The development of biofilms and the role they play in corrosion and deposition processes may be the most misunderstood and underestimated factor in the treatment of cooling water and other industrial water systems. Ask any water treatment professional about calcium carbonate scale or to explain the major attributes of a typical corrosion cell, and you may get a reasonable explanation provided with some confidence. Ask about biofilms, and you will get a different response. This review is designed to provide a basic understanding of what biofilms are, the problems they can cause, and what might be done to deal with them.
What is a biofilm?
Simply stated, a biofilm consists of microbial cells (algal, fungal, or bacterial) and the extracellular biopolymer they produce. Bacterial biofilms are of most concern in industrial water systems, since they are generally responsible for the fouling of heat transfer equipment. This concern is due, in part, to the minimal nutrient requirements that are required for many species to grow. One should keep in mind that the more nutrients available in the form of useable organic carbon, the greater the diversity and numbers of organisms that can be supported. When dealing with cooling towers and spray ponds, algal biofilms also are a concern. Not only will algal biofilms foul distribution decks and tower fill, but algae will also provide nutrient (organic carbon) that will help support the growth of bacteria and fungi. Algae do not require organic carbon for growth, but instead utilize CO2 and the energy from light to manufacture carbohydrate. Thus, a cooling water system with little organic carbon can generate its own through the growth and dispersal of algal cells.
Microorganisms can be found in both the bulk water and on the surfaces of industrial water systems. Bacteria attach to surfaces by proteinaceous appendages referred to as fimbriae (Fig. 1). Once a number of fimbriae have attached the cell to the surface, they make detachment of the organism very difficult. One reason bacteria prefer to attach to surfaces is that the organic molecules adsorbed there provide nutrient. Once attached, the organisms begin to produce material termed extracellular biopolymer, or “slime” for short. The amount of biopolymer produced can exceed the mass of the bacterial cell by a factor of 100 or more. The extracellular polymer (EPS)
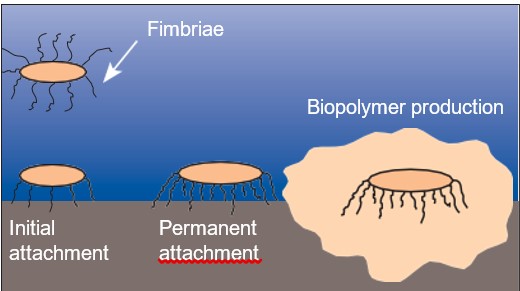
produced may provide a more suitable protective environment for the survival of the organism. The fimbriae, the EPS, and even the flagella may be involved in cell.
Figure 1. Attachment of bacteria to surfaces. attachment.
The extracellular biopolymer consists primarily of polysaccharide, protein, and water. In fact, biofilms are generally greater than 90% water. The polysaccharides produced vary depending on the species, but are typically made up of repeating oligosaccharides, such as mannose, gulose, glucose, galactose, xylose, and others. An often cited example of a bacterially produced biopolymer is xanthan gum, produced by Xanthamonas campestris (Fig. 2). This biopolymer is produced for use as a thickening agent in a variety of food and consumer products. Gellation of some biopolymers can occur upon addition of divalent cations, such as calcium and magnesium. The electrostatic interaction between carboxylate functional groups on the polysaccharide and the divalent cations results in a bridging effect between polymer chains. Bridging and crosslinking of the polymers help to stabilize the EPS, making the biofilm more resistant to shear.
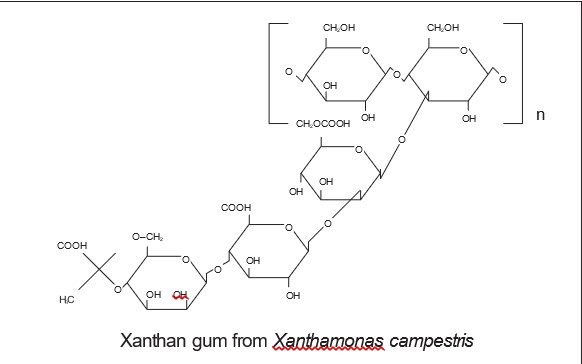
Figure 2. Structure of xanthan gum.
Problems associated with biofilms
Once bacteria begin to colonize surfaces and produce biofilms, numerous problems begin to arise, including reduction of heat transfer efficiency, fouling, corrosion, and scale. When biofilms develop in low flow areas, such as cooling tower film fill, they may initially go unnoticed since they will not interfere with flow or evaporative efficiency. After time elapses, the biofilm becomes more complex, often with filamentous development. This matrix will accumulate debris that may impede or completely block flow (Fig. 3).
Figure 3. Stages of fouling development.

Biofilm structure is often imagined as a coating of microbial cells and biopolymer that is spread evenly across a surface. In reality, biofilm structure is much more complex. Biofilms may be patchy and highly channelized, allowing nutrient-bearing water to flow through and around the matrix.
Algal biofilms may foul cooling tower distribution decks, tower fill, and basins. When excessive algal biofilms develop, portions may break loose and can be transported to other parts of the system, causing blockage as well as providing nutrient for accelerated bacterial and fungal growth. Biofilms can cause fouling of filtration and ion exchange equipment. Figure 4 shows a 75 micron cartridge filter fouled with biofilm. In photograph A (300X), the filter fibers are shown to be fouled with an unknown material. Closer examination of the foulant exhibited in photograph B (9000X) shows the foulant to be bacterial cells and extracellular biopolymer.
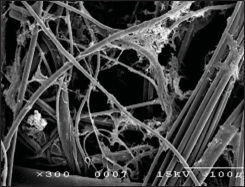
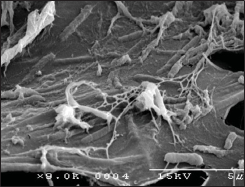
Bacterial biofilms may also foul heat exchange equipment. Bacterial fouling of heat exchangers can occur quickly due to a process leak or influx of nutrient. The sudden increase in nutrient in a previously nutrient-limited environment will send bacterial populations into an accelerated logarithmic growth phase, which can result in a rapid accumulation of biofilm. The biofilms that develop will then interfere with heat transfer efficiency. Table 1 demonstrates the thermal conductivity of a variety of deposit-forming compounds in contrast to biofilm. A lower number indicates a greater resistance to heat transfer.1
Table 1. Thermal conductivity comparison of deposit-forming compounds and biofilm
Substance | Thermal conductivity (W m–1 K–1) |
CaCO3 | 2.6 |
CaSO4 | 2.3 |
Ca3(PO4)2 | 2.6 |
Fe2O3 | 2.9 |
Analcite | 1.3 |
Biofilm | 0.6 |
Deposits in the form of biofilm, and biofilm with entrapped suspended debris, are generally easy to comprehend; but biofilms may often lead to the formation of mineral scales, as well. Calcium ions are fixed into the biofilm by the attraction of carboxylate functional groups on the polysaccharides. In fact, divalent cations, such as calcium and magnesium, are integral in the formation of gels of some extracellular polysaccharides. These calcium ions are fixed in place by the biofilm at the heat transfer surface, thereby making them more readily available to react with carbonate or phosphate anions that are present, also. This would then provide nucleation or crystal growth sites that would not normally be present on a biofilm-free surface. Additionally, biofilms may entrap precipitated calcium salts and corrosion byproducts, which will act as crystal growth sites, from the bulk water.
A typical biofilm-induced mineral deposit, with which we all are familiar, is the calcium phosphate scale that the dental hygienist removes from our teeth. When biofilms grow on tooth surfaces, they are referred to as plaques. If these plaques are not continually removed, they accumulate calcium salts, mainly calcium phosphate, to form tartar (scale) (Fig. 5 & 6). One could make a comparison between rinsing your mouth twice daily with an antiseptic mouthwash to control plaque and feeding microbiocides and biodispersants to control biofilm-related deposition in heat exchangers. If biofilms in heat exchangers are not controlled, then, like dental plaques, the mineral scale may deposit.
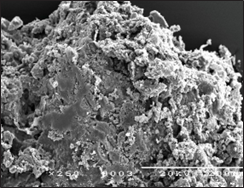
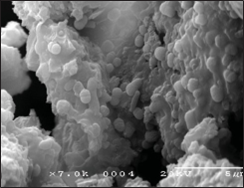
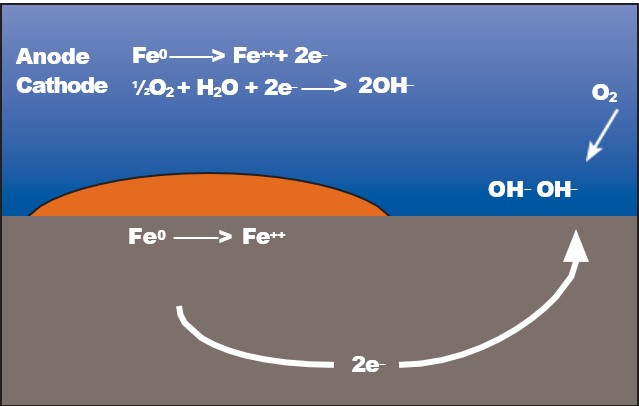
The growth of bacteria and formation of biofilms may also result in another problem: that of corrosion. Microbiological corrosion may be defined as corrosion that is influenced by the growth of microorganisms either directly or indirectly To understand microbiologically influenced corrosion, or MIC for short, it helps to have a basic understanding of corrosion chemistry.
This document is not intended to provide that information, but some water treatment training manuals will.In essence, corrosion occurs on a metal surface due to some inherent or environmental difference between one area on that surface and another. These differences will create anodic and cathodic areas, setting up a basic corrosion cell (Fig. 7). The anode is the area at which metal is lost. The electrons are given up by the metal flow to the cathode to be consumed in a reduction reaction. Microbiological corrosion is electrochemical corrosion, where in some manner, the presence of the microorganisms is having some influence in the creation or acceleration of corrosion processes.
Microorganisms can influence corrosion in a variety of ways. Formation of localized differential cells, the production of mineral and organic acids, ammonia production, and sulfate reduction are just a few of the mechanisms by which bacteria, fungi, and algae can influence corrosion. The formation of localized cells is the primary mechanism of corrosion caused by iron-oxidizing bacteria, such as Gallionella sp., Arthrobacter siderocapsulatus, and Pseudomonas putida.2 When iron- and manganese-oxidizing organisms colonize a surface, they begin to oxidize available reduced forms of these elements and produce a deposit. In the case of iron-oxidizing organisms, ferrous iron is oxidized to the ferric form (Fe++ —> Fe+++ + 1e–), with the electron lost in the process being utilized by the bacterium for energy production. As the bacterial colony becomes encrusted with iron (or manganese) oxide, a differential oxygen concentration cell may develop, and the corrosion process will begin. The ferrous iron produced at the anode will then provide even more ferrous iron for the bacteria to oxidize. The porous encrustation (tubercle) may potentially become an autocatalytic corrosion cell, or may provide an environment suitable for growth of sulfate-reducing bacteria. Figs. 8, 9, and 10 illustrate the stages of localized corrosion cell development resulting from the growth of iron-oxidizing bacteria.
Corrosion may also develop when localized cells are formed, due simply to biofilms developing on metal surfaces. The oxidation of iron or manganese is not a requirement for the development of a localized corrosion cell.
Numerous factors can contribute to localized corrosion on metal surfaces. The production of ammonia by the reduction of nitrates, or nitrites, may lead to severe localized loss on copper-based metallurgy. The production of organic acids, such as acetic, butyric, or citric acid, may help solubilize protective metal oxide films. Inorganic acid, such as sulfuric acid produced by Thiobacillus sp., can also have detrimental effects. As biofilms develop, they will eventually achieve a thickness at which oxygen concentration is either very low or completely excluded. At a thickness of just 200 microns, the oxygen concentration within the biofilm is reduced to near zero ppm. When this occurs, facultative and obligate anaerobes (such as Desulfovibrio sp. and Clostridium sp.) can flourish.
Anaerobic sulfate-reducing bacteria (e.g. Desulfovibrio) are the bacteria most often considered when discussing microbial corrosion. These organisms can seek out and colonize areas deficient in oxygen, such as those found within porous corrosion tubercles, within biofilms, and under debris. These bacteria are responsible for rapid and severe metal loss in industrial water systems. This type of corrosion is easily recognizable from the characteristicsulfide byproduct present within the corrosion cell. Sulfate- reducing bacteria primarily cause corrosion by utilizing the molecular hydrogen produced at the cathode, thereby depolarizing it (Fig. 11). Since the rate of corrosion is under cathodic control, removal of cathodic reduction products will increase the rate of corrosion. Systems that are sulfate-limited will have less of a tendency to be attacked by SRB.
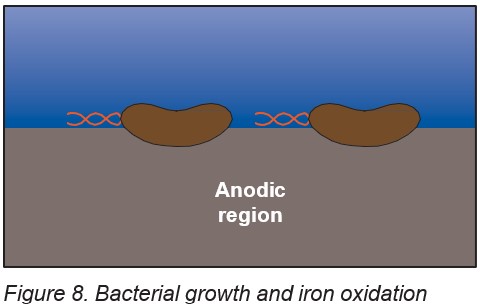
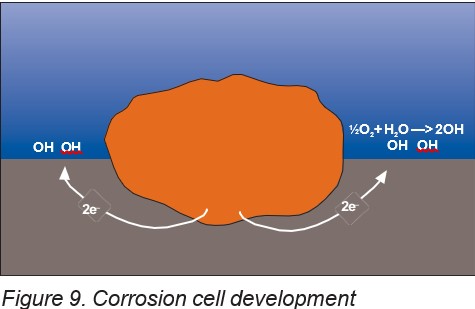
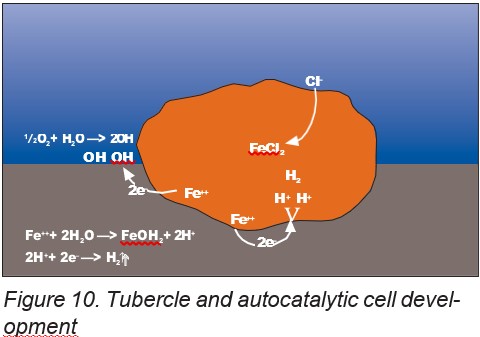
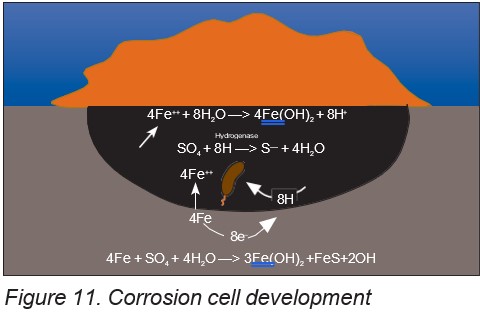
Biofilm control
It can be seen that the growth of bacteria on surfaces in cooling and process water systems can lead to significant deposit and corrosion problems. Once this is unders tood, then the importance of controlling biofilms becomes quite clear. Too often, micro biological control efforts focus only on planktonic counts, that is the number of bacteria in the bulk water. While some useful data may be gathered from monitoring daily bacterial counts, monthly or weekly counts are simply time wasted and have little meaningful use. Planktonic counts do not necessarily correlate with the amount of biofilm present. In addition, planktonic organisms are not generally responsible for deposit and corrosion problems. There are a few exceptions, such as a closed loop system, where planktonic organisms may grow, degrade corrosion inhibitors, and excrete acids which depress pH. Systems with low planktonic counts may have a significant biofilm problem, and vice versa. Therefore, efforts should focus on general biofilm control. Biofilms can be controlled through the use of microbiocides, biodispersants, and by limiting nutrients. Microbiocides, both oxidizing and nonoxidizing, can be effective in overfall biofilm control when applied properly.5 The oxidizing microbiocides, such as chlorine, bromine, chlorine dioxide, peracetic acid, and ozone, can be extremely effective in destroying both the EPS and the bacterial cells. When using oxidizing microbicides, one must be sure to obtain a sufficient residual long enough to effectively oxidize the biofilm. Unfortunately, there are those who are overly concerned with the corrosive nature of the oxidizing microbiocides and fail to apply the needed residual oxidant required to control biofilm. Low residual oxidant levels may significantly reduce planktonic counts, but may not be sufficient to control biofilm.
The level of oxidant and duration required will vary from system to system. Low-level, free-residual oxidant (FRO) will kill planktonic bacteria, yeast, mold, and protozoa. However, medium to high dosages of FRO are needed to oxidize biofilms. Algal mats, particularly, require high dosages of FRO. It is generally more effective to maintain a higher residual for a duration of several hours than it is to continuously maintain a low residual. Continuous low-level feed may not achieve an oxidant concentration sufficient to oxidize the polysaccharide and expose the bacteria to the oxidant. Another misconception is with the use of chlorine at alkaline pH (> 8.0). It is often thought that chlorine is ineffective for controlling microorganisms at elevated pH. This is only half true. Certainly, the hypohalous acid form of chlorine (HOCl) is more effective at killing cells than the hypohalite form (OCl–). However, the hypohalite is actually very effective at oxidizing the extracellular polysaccharide and the proteinaceous attachment structures. Therefore, the use of chlorine in alkaline cooling waters can still be extremely effective when applied properly. This is especially true when combining chlorine with bromine or with a compatible nonoxidizing microbiocide such as a polyquat. When this is done, one achieves both oxidation of the extracellular material and sufficient kill of the microorganisms
Bromine compounds, such as NaBr used to generate free residual HOBr and organobromine biocides, are very effective oxidizing biocides. Bromine is extremely deadly to microbes, and the kinetics of the kill reactions is very quick.
JC 9465 is new oxidizing chemical that is more effective than chlorine. JC 9465 was proven highly effective as a single, standalone biocide in a variety of applications where chlorine alone failed, including paper, cooling water, food and beverage, utilities, and oil and gas.
In Chart No.1, we see that electrochemical voltage is a means to determine the strength of an oxidizing biocide. Ozone which a common and well-known oxidant is 2.07 volts, sodium hypochlorous acid (HOCL) is 1.36 volts and JC 9465 is 2.7 volts-2.8 volts which indicates more energy for quicker disinfections.
Chart No. 1
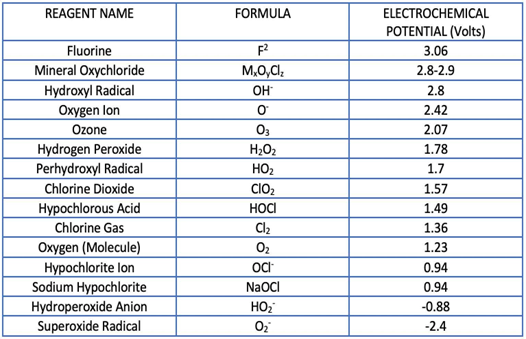
Chart No.2 indicates the higher the voltage the quicker disinfection occurs. JC 9465 has demonstrated quicker disinfection as a single oxidant and was proven highly effective in a variety of applications where chlorine alone failed, including paper, cooling water, food and beverage, utilities, and oil and gas. Chart No. 2
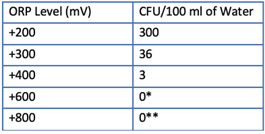
*Water Disinfection
**Water Sterilization
Certain nonoxidizing microbiocides are also effective in controlling biofilm. Effective control is greatly dependent on the concentration of the product feed, frequency of addition, dosage fed, and resistance of the incumbent population to the product fed. Control cannot generally be achieved by once-a-week additions, as is common in “full service” applications. Typical application for effective control may include a slug addition of product 2 to 5 times a week. As with oxidizing microbiocides, frequency and dosage will depend on the system conditions. It is generally most effective to alternate nonoxidizing microbiocides at every addition to ensure broad- spectrum control. Most nonoxidizing microbiocides will have little effect in destroying the extracellular poly saccharide found in the biofilm. However, many of these microbiocides may be able to penetrate and kill bacteria found within the biofilm, resulting in decreases in the population and weaknesses in the biofilm structure. Thus, combining the use of nonoxidizing and oxidizing microbiocides is a very effective means of controlling biofilm. When using a nonoxidizing microbiocide in conjunction with an oxidizing agent, there should be a slight to no residual oxidant concentration present in the system at the time of addition. Sufficient time should be allowed for the nonoxidizing microbicide to work before resuming oxidant feed unless an oxidant-compatible microbiocide is being used (i.e., polyquat). Using combination biocides has proven very effective in killing unwanted bacterial species.3 Application of synergistic biocides, in particular, can give improved biocide performance against harmful bacteria.4,7,8
Biofilm control programs can be made more effective through the use of biopenetrant/dispersant products. Products that penetrate and loosen the biopolymer matrix will not only aid in sloughing the biofilm but will also expose the microorganisms to the microbicides. Biodispersants are especially effective when dealing with systems that have a high TOC loading and a tendency to foul. These products are typically fed in slug additions prior to microbiocide feed. Low-level continuous feed may also be effective. However, in some cases low- level continuous feed may not be as effective since it may not reach a certain threshold amount required to produce the desired effect. Recent developments in biodispersant technology are making this approach more effective and popular than ever before. Enzyme technologies that will break down the extracellular polysaccharides and degrade the bacterial attachment structures (fimbriae) are currently being developed and patented. These technologies provide biofilm control where microbicide use is environmentally limited or restricted or provide a means of quickly restoring fouled cooling water systems to a clean, efficient operable state. Recent applications in the field proved that enzyme technologies improve the efficiency of biocontrol programs. The importance of biofilm control must not be taken lightly. It is the fundamental basis for controlling a high percentage of deposition and corrosion problems in process and cooling water systems. Once these fundamentals are understood, effective treatment strategies can be developed.
References
1 Characklis, W.J., M. H. Turakhias, and N. Zelver. 1990. “Transport and Interfacial Transfer Phenomena,” in Biofilms, ed. by W.J. Characklis and K.C. Marshall, New York: John Wiley and Sons, p. 316.
2 Chun, J., M-S Rhee, J-I Han, and K.S. Bae. 2001. Arthrobacter siderocapsulatus Dubinina and Zhdanov 1975AL is a later subjective synonym of Pseudomonas putida (Trevisan 1889) Migula 1895AL. Intl. J. Systematic and Evolutionary Microbiology. 51: 169 –170.
3 Wiatr, C. L. 2002. Detection and eradication of a non- legionella pathogen in a cooling water system. The Analyst. Assoc. of Water Technologies. IX: 38–48.
4 Wiatr, C. L. 2002. Detection and Eradication of a non- legionella pathogen in a recirculating cooling water system. Amer. Soc. for Microbiology. Q-132 (Salt Lake City, UT).
5 Sumrall, T., C. Wiatr, J. Chalut. 2015. Systems and Methods for Generating Haloamines and Applications Thereof in Oil and Gas Operations. U.S. Patent 2015/0329387A1.
6 Sumrall, T., C. Wiatr, J. Chalut. 2016. Systems and Methods for Generating Haloamines and Applications Thereof in Oil and Gas Operations. Patent WO2016010621A1.
7 Wiatr, C. L. 2016. Bacterial Resistance to Antimicrobials in Cooling Water Systems. Part 1. The Analyst. 23(2): 11-16.8Wiatr, C. L. 2016. Bacterial Resistance to Antimicrobials in Cooling Water Systems. Part 2. The Analyst. 23(3): 12-23.
Leave A Comment